INTRODUCTION
Currently, there is a growing search for materials that are ecologically more aligned with the environmental sustainability model, in the same way as environmental awareness grew in the face of the destruction of the environment caused by man. For this reason, vegetable fibers are being widely used, in addition to having good mechanical properties, low cost, low density, it is a biodegradable material with wide availability, etc. According to Marinelli et al. (2008), all these aspects have turned plant fibers into a potential substitute for synthetic fibers. In this work, it was carried out the study on the mechanical resistance of hybrid composite materials on polyester matrix, with graphene nanoparticles and using the curauá fiber, which is the vegetable fiber of greater mechanical properties and lower density among all plant fibers (Chegdani et al., 2017). The orthophthalic unsaturated polyester resin was used as a matrix in the work. This type of resin has been widely used for the production of compounds due to its mechanical properties and versatility in its applications, it has low water absorption and ability to cure at room temperature and it has transparency (Sanchez et al., 2010).
It is known from previous works reported in the literature, that the addition of particles of high rigidity in the polymer improves the properties of the compound such as mechanical strength and modulus of elasticity/rigidity avoiding fiber failures in the transverse direction of the laminate, by the placement of reinforcement particles in the interlaminar region making difficult the propagation of the cracks, in such a way that their performance is visibly improved (Abot et al., 2007, 2008). Recently, it has been verified that graphene oxide can be considered more appropriate than graphene itself to be used as fillers in polymeric nanocomposites. It is mainly due to the presence of oxygenated groups bound on the surface, such as hydroxyl, epoxy, carbonyl and carboxyl and possible chemical functionalization of graphene oxide in the direction of providing more affinity with the matrix, resulting in a compound with higher thermomechanical performance (Jajam y Tippur, 2012).
According to Pathak et al. (2016), in a recent study on the effect coming from the addition of graphene oxide in the compound based on epoxy resin with carbon fiber, it was clearly revealed the improvement of mechanical properties, especially in the flexural strength, which had an increase of 66% and the modulus of elasticity of 70% with the addition of 0.3 wt% of graphene oxide. This improvement in producing a hybrid nanocomposite is based on a solid physical and chemical interaction of the dispersed phase with the matrix and that the reinforcing particles in compounds must have peculiar characteristics, such as functional groups with chemical affinity, which increase the approximation between the matrix and the load (Kumar et al., 2014; Kumari et al., 2016).
In common with all natural fibers, cellulose is one of the main components and its importance is to provide mechanical strength to the fiber. Cellulose is a polysaccharide that has in its composition the elements carbon, oxygen and hydrogen. Cellulose has two polymorphous forms type I and II in which the first corresponds to the arrangement of the cellulose chain as found in nature, with greater resistance than the polymorphic form II which is obtained through the process of mercerization or regeneration of the native cellulose (Zuber et al., 2012). In Figure 1 this transformation is schematized.
Chemical treatments of fiber surface modification are widely used to optimize fiber / matrix bonding. Parameters such as type of treatment, concentration of the solution and immersion time of the fibers in the solutions are very important elements to achieve the optimal mechanical behavior of the compounds (Yu et al., 2010 and Porras et al., 2016). For this reason, in this work the treatment with sodium hydroxide with different concentrations and different immersion times was studied.
In this work, several parameters were studied, such as fiber content and graphene oxide content, as well as chemical treatment parameters such as concentration of sodium hydroxide solutions and the immersion time of the fibers in those solutions. Taguchi Method was used to integrate the study of all the parameters and maximize the performance of the composite material. The Taguchi method has been used in several works with very good reliability results, optimization in the analysis of the results and economy in the design of the experiment integrating the processing parameters (Kumar et al., 2014; Kumari et al., 2016). This method allows the optimization of the materials performance by adjusting the processing parameters through a robust experimental design that has the ability to analyze many parameters with fewer experiments than in traditional experiments. In fact, this technique, widely used to improve the quality of articles or products manufactured, was recently implemented in the manufacture of green compounds, such as studying and improving the wear behavior of natural fiber compounds and optimizing the mechanical properties (Biswas y Satapathy, 2010; Raghavendra et al., 2012). The Taguchi method is a powerful analysis tool to conduct experimental work involving the study of multiple parameters. The method uses orthogonal matrices to determine the minimum number of experiments necessary to obtain sufficient information for determining optimal values of the evaluated factors (Patnaik et al., 2010; Hussain et al., 2011; Pathak et al., 2016).
MATERIALES Y MÉTODOS
The material used for the matrix was the orthophthalic unsaturated polyester resin produced from the orthophthalic acid supplied by the Redilease Company in the City of São Paulo Brazil. Curauá fibers were supplied by SENAI from the City of Santarém Pará State of Brazil and the Graphene oxide was obtained in the Semi-Industrial Laboratory of the Faculty of Chemistry of the University of São Paulo.
The factors studied in this work were the fiber content of curauá, the concentration of the sodium hydroxide solution for the treatment of the fiber, the exposure time of the fibers in each solution and the manometric particle content of graphene oxide. The fiber contents used were 10, 20 and 30 (wt%), the concentrations of sodium hydroxide used were 2.5, 5 and 10% (wt%), the inmersion times of the fibers in the corresponding solutions were 2, 4 and 8 hours and the amounts of graphene oxide used were 0.1, 0.5 and 1% (wt%). The objective of the work was to determine the influence of these factors on the mechanical strength of the compound given by the Traction and Flexion Effort.
First, an experimental design was made using the Taguchi Method according to the MINITAB17 software, with which the number of test bodies was greatly reduced. Table 1 shows the design of Taguchi L9 (34) (orthogonal ordering) determined by MINITAB 17 Software. L9 means 9 runs or 9 test bodies. The term 34 means 4 factors with 3 levels each factor. If the complete factorial design were used, it would have 81 runs. The L9 array (34) is a fraction of the complete factorial design that corresponds to only 9 runs. In this orthogonal array, factor levels are weighted in the same way throughout the design (Yu et al., 2010). The columns of the table represent the control factors, the rows of the table represent the runs (combination of factor levels) and each cell of the table represents the level of the factor for each run as shown in table 2.
TABLE 1 Factors and levels of the experiments according to Taguchi's design (34), MINITAB 17
Factor | Level 1 | Level 2 | Level 3 |
---|---|---|---|
A: Fiber quantity (wt%) | 10 | 20 | 30 |
B: Concentration of the sodium hydroxide solutions (wt%) | 2.5 | 5 | 10 |
C: Inmersion time of fiber (h) | 2 | 4 | 8 |
D: Quantity of nanometric particles of graphene oxide (wt%) | 0.1 | 0.5 | 1 |
TABLE 2 Representation of the Taguchi matrix (L9)
N | A | B | C | D |
---|---|---|---|---|
1 | 1 | 1 | 1 | 1 |
2 | 1 | 2 | 2 | 2 |
3 | 1 | 3 | 3 | 3 |
4 | 2 | 1 | 2 | 3 |
5 | 2 | 2 | 3 | 1 |
6 | 2 | 3 | 1 | 2 |
7 | 3 | 1 | 3 | 2 |
8 | 3 | 2 | 1 | 3 |
9 | 3 | 3 | 2 | 1 |
The experimental part began with the preparation of the sodium hydroxide solutions and the placement of the fibers in the corresponding solutions, which were removed from the solutions according to the planned times. After removed, the fibers were well rinsed in distilled water until a PH 7 was obtained and then they were dried in an oven at 60 ° C for 24 hours and at 90 ° C for 2 hours. Later to know the effectiveness of the chemical treatments carried out, fibers of each group were observed through the Electronic Scanning Microscope. The use of scanning electron microscopy was carried out with the objective of observing the magnitude of the effect of the alkaline treatment performed on natural fibers, given by the separation of the cellulose fibrils and the surface roughness of the fiber, which should allow a greater adhesion between fiber and matrix (Satyanarayana et al., 2007). A Philips XL-30 Electronic Scanning Microscope with secondary electron detector was available at the Laboratory of the Metallurgical and Materials Engineering Department of the University of São Paulo.
Subsequently, X-ray diffraction was performed in each corresponding fiber group, the objective of the analysis with X-ray diffraction was the determination of the phases present in the samples of curauá fibers treated with different treatment conditions and to determine the crystallinity in each type of fiber. The analyses were performed on a Rigaku brand X-ray diffractometer available in the Laboratory of the Metallurgical and Materials Engineering Department of the University of São Paulo, with a CuKα radiation source. The diffraction conditions were step equal to 0.04 °, time per step of 4 seconds for the interval of 2θ between 0 the 40 °.
To perform the mechanical tests, specimens were manufactured by delamination in an open mold with the dimensions according to ASTM D 638 (I) [18] with compositions that varied according to the experiments planned in the Taguchi Matrix. The mechanical tensile and bending tests were carried out in the KRATOS® 5002 traction machine available in the Laboratory of the Department of Metallurgic and Materials Engineering of the University of São Paulo.
RESULTS
Figure 2 shows the micrographs of the treated and untreated curauá fibers where the effect of the NaOH treatment on the morphology of the fibers can be observed (Figures 2b and 2c) in comparison with the untreated fibers (Figure 2a).
Studies with lignocellulosic materials, among them on the curauá fiber itself, point to a reflection in the crystallographic plane (002) with greater intensity, which corresponds to the rede planes of the glycosidic rings that are the densest structure of type I cellulose corresponding to the angle 2θ = 22º. Two other peaks of lower intensity in 16º and 35º are also found next to it, which have their respective planes (101) and (040). With the results obtained in the diffractometer, the crystallinity indexes of the curauá fibers were calculated using the Segal empirical method that provides an approximate value of crystallinity (Lengowski et al., 2013). The obtained crystallinity results are shown in table 3.
In Figure 2, the micrographs of the treated and untreated fibers are shown.
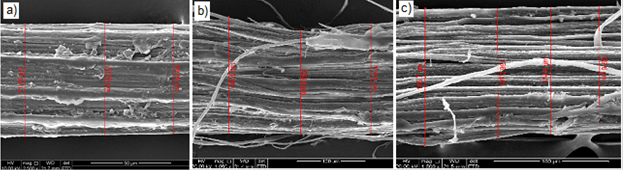
FIGURE 2 Micrographs of the fibers: a) without treatment, b) with treatment of 5% NaOH and immersion time of 4 hours c) with treatment of 10% NaOH and immersion time of 4 hours.
In Figure 3, the difframograms of the fibers without treatment and with NaOH treatment of 5% and 10% with inmersion time in the solution of 4 hours are shown. In Table 3, the values of crystallinity obtained in each corresponding fiber can be observed.
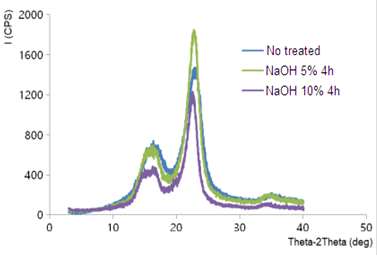
FIGURE 3 Diffractograms of the fibers without treatment and with treatment of 5% and 10% for 4 hours.
TABLE 3 Result of the Crystallinity Index (%) as a function of the concentration of sodium hydroxide and the exposure time of the fibers in the solutions
NaOH concentration (wt%) / inmersion time (h) | 2 h | 4 h | 8 h |
---|---|---|---|
0 | 32 | 32 | 32 |
2,5 | 35 | 36 | 38 |
5,0 | 38 | 48 | 46 |
10,0 | 42 | 47 | 46 |
The tensile test was performed with an initial load cell of 2000kgf with a speed of 5 mm / min. The bending test was carried out with an initial load of 2500kgf with a speed of 3 mm / min. The strength and speed parameters were recorded by transmission to the computer by the LYNX® ADS 2000 data system. The values obtained for maximum tensile and flexural strength for the pure polyester resin can be found in Table 4 and the values obtained from maximum tensile and flexural strength for the different materials manufactured according to the Taguchi experiment design are found T 5.
TABLE 4 Results obtained in the experimental tests for pure Polyester resin
Fiber (wt%) | NaOH (wt%) | Time (h) | Graphene oxide (wt%) | Tensile stress (Mpa) | Elasticity module (Mpa) | Elongation (%) | Flexion stress (Mpa) |
---|---|---|---|---|---|---|---|
0 | 0 | 0 | 0 | 25.95±0.43 | 293.78 | 20.90 | 19±0.23 |
To determine the optimal configuration of the parameters to maximize the tensile and flexural properties, the experimental data were transformed into a signal-to-noise ratio (S/N) for the analysis. As the objective of Taguchi's analysis is to maximize tensile strength and flexural strength, the S/N ratio chosen at the MINITAB 17 software was as “the larger the better” (LBT), which is calculated as a logarithmic transformation of the loss function, as shown in Equation 1, whose values obtained through the MINITAB 17 software for each case are found in Table 5.
Where:
η |
- Signal noise ratio (dB) |
n |
- number of observations |
Y |
- response values obtained in the tests (output). |
TABLE 5 Results obtained in the experimental tests and corresponding analysis of the Signal / Noise Ratio obtained by the MINITAB Software 17
N | A | B | C | D | Tensile stress (Mpa) | Ratio S/N (dB) | Flexion stress (Mpa) | Ratio S/N (dB) |
---|---|---|---|---|---|---|---|---|
1 | 10 | 2.5 | 2 | 0.1 | 30.53±0.33 | 29,69 | 24.32±0.28 | 27,71 |
2 | 10 | 5.0 | 4 | 0.5 | 36.32±1.47 | 31,20 | 32.98±0.24 | 30,36 |
3 | 10 | 10.0 | 8 | 1.0 | 33.34±2.4 | 30,45 | 28.89±0.65 | 29,21 |
4 | 20 | 2.5 | 4 | 1.0 | 37.12±1.44 | 31,39 | 30.08±0.56 | 29,56 |
5 | 20 | 5.0 | 8 | 0.1 | 44.56±0.87 | 32,97 | 39.13±1.41 | 31,85 |
6 | 20 | 10.0 | 2 | 0.5 | 43.45±0.98 | 32,75 | 37.32±2.52 | 31,43 |
7 | 30 | 2.5 | 8 | 0.5 | 45.32±0.56 | 33,12 | 40.24±0.64 | 32,09 |
8 | 30 | 5.0 | 2 | 1.0 | 48.76±0.79 | 33,76 | 43.54±1.13 | 32,77 |
9 | 30 | 10.0 | 4 | 0.1 | 46.86±1.56 | 33,41 | 42.36±2.12 | 32,53 |
Results of Tensile and Flexural Strength Tests
Table 5 shows the results of Taguchi's experimental design, presenting the average tensile and flexural strength values obtained in the experimental tests and their respective S / N values for each test. The average tensile strength of the 9 tests was 40.69 MPa with an average signal / noise ratio of 32.08 dB and the average resistance to bending was 35.42 MPa, with an average signal / noise ratio of 30.84 dB. The best traction behavior was found for the No. 8 test and the lowest for the N.1 test, which also coincides with the best and worst bending results.
Results of the Analysis of the Mean Values of Resistance and of Relation Signal / Noise (S / R)
In order to analyze the effect of each factor on tensile and flexural properties, the analysis of the means (ANOM) was performed through the MINITAB 17 software. This analysis involves calculating the average of the response criteria for each factor level. The highest average response is selected to determine the optimal level for each factor. Figures 4 and 5 summarize the ANOM analysis results for tensile and flexural strength, respectively.
The ANOM means analysis was also performed for the Signal / Noise ratio (S / N). Tables 6 and 7 show the results of the ANOM analysis for S / N corresponding to tensile and flexural strength, respectively. The optimum factor level adjustment points were those with the highest S / N ratio. This analysis shows the best response with the least effect due to noise. The optimum combination of the process parameters for the tensile and maximum bending properties was A3, B2, C3, D2. This result coincides with the analysis of the ANOM averages made for the means of tensile and flexural strength.
TABLE 6 Average values of the S / N ratio (dB) of each factor corresponding to the tensile stress results
Level | A: Fiber quantity (wt%) | B: Sodium hydroxide (wt%) | C: Inmersion time (h) | D: Graphene oxide (wt%) |
---|---|---|---|---|
1 | 30,44 | 31,4 | 32,06 | 32,02 |
2 | 32,37 | 32,64 | 32 | 32,35 |
3 | 33,43 | 32,20 | 32,18 | 31,86 |
DISCUSSION
From the micrographs obtained, the effect of the NaOH treatment was observed, where the extraction of hemicellulose and lignin and changes in the chemical composition of the fibers are noted, including its morphological aspect in which, different cellulose fibrils, are clearly observed. The structural changes begin to be more expressive in the same proportion in which the treatment time is prolonged. These changes are of fundamental importance especially in polymeric compounds reinforced with vegetable fibers, becuse they exert an influence on the mechanical performance since the structural alterations are advantageous in the sense of facilitating the interpenetration of the polymeric matrix in internal parts of the fibers, improving this way, the adhesion and therefore, the mechanical strength of the compound.
On the diffractogram made, as it can be seen in Figure 3 and Table 3, the efficiency of the treatment of 5% in relation to the fibers without treatment and even that of 10% can be verified. It can be seen that the second peak of the curve corresponding to the concentration of 5% is much more intense than the other curves announcing that there is a higher concentration of cellulose phase type I than in the other samples. It can be seen that at the peak of the 10% treatment there is a change with the emergence of two peaks, which, according to the literature, is a consequence of the transformation of the cellulose phase from type I to type II which decreases the mechanical strength of the fiber (Lengowski et al., 2013). Therefore, the excess delignification can damage the fiber itself because it destroys a part of the crystalline structure, and, consequently, reduces the reinforcement capacity to the matrix.
According to the results, the increase of the curauá fiber quantity up to 30% in weight increased the values of tensile and flexural strength due to greater interaction between the matrix and the fiber, having in this way, a more uniform distribution of stresses through the body of the compound. From previous works it is known that increases in fiber content greater than 30 wt% do not introduce increases in the mechanical strength of the compounds due to the low impregnation of the fiber / matrix interface, which cause the delamination of the compound (Mohanty et al., 2000). The chemical treatment applied to the fibers was more effective when solutions of medium concentration and long treatment times were used. This result of chemical treatment is consistent with the recommendations of the literature (Yu et al., 2010), since they show high chemical concentrations and reduce the mechanical properties due to the depolymerization of the cellulose and an excessive process of delignification of the fiber (Mishra et al., 2003). However, long treatment times are necessary to remove impurities and waxes. According to the mean main effects graphs (ANOM), the treatment time has a greater effect on the mechanical performance of the compound, while high concentrations of sodium hydroxide decrease the tensile and flexural strength.
Through the graph of analysis of the means (ANOM), it can be seen that the concentration of the synthetic load more appropriate in the experiment was 0.5 wt% and that for higher increases in graphene oxide the compound shows losses in mechanical performance. That can be justified by the agglutination between the loads. It harms a good homogenization and originates fragilizing points.
CONCLUSION
Through the results of the work it is shown that the use of two types of reinforcement in the same polymeric matrix resulted in a considerable increase in the resistance of the compound in relation to the pure polyester matrix. An average tensile strength of 40.69 MPa was obtained, which represents 156% in relation to pure polyester and an average flexural strength of 35.42 MPa, which represents 186% in relation to the flexural strength of pure polyester .
Through the results obtained, it was possible to verify the positive effect of the increase of the curauá fiber up to 30 wt%. Also, the alkaline treatment of the fiber had an increase in the mechanical resistance of compounds in polyester resin matrix for medium concentrations and prolonged immersion times. In addition, the increase of graphene oxide in concentrations up to 0.5% p contributed to the increase in the limit of tensile and flexural strength of the compound.