Introduction
The current pandemic Coronavirus Disease-19 (COVID-19) is caused by the sever acute respiratory syndrome coronavirus 2 (SARS-CoV-2), which belongs to the Coronaviridae family and the Betacoronavirus genus that affects human beings.1 SARS-CoV-2 infection originated in Wuhan, China in late December 2019. Since then, the virus has infected millions of people worldwide.2 As of November 10, 2022, confirmed cases of COVID-19 were 630,601,291 and 6,583,588 related deaths were reported throughout the world. Also, a total of 12,885,748,541 vaccine doses had been administered.3 Most vaccine doses have been administered in China (3,452,957,751) followed by India (2,170,901,372) (Table 1). SARS-CoV-2 induces respiratory system complications; symptoms include fever, myalgia, cough, and fatigue. Researchers reported the viral genetic and protein sequence in time and strategies have been established to mitigate the pandemic through the development of effective drugs and vaccines.4,5 Information about viral structure proteins, such as main protease (Mpro), spike proteins (S protein) and RNA dependent RNA polymerase (RdRp), would help to design drugs against SARS-CoV-2. Additionally, various small molecular drugs and vaccine are being developed for treatment of COVID-19.6,7
According to literature, in 1918, during influenza pandemic, the second phase of outbreak was more severe than the first one. Scientists predicted the coronavirus outbreak a decade ago, but the world was not prepared to handle the pandemic.8 SARS-CoV-2 has a long incubation period (range 2-14 days), easy and fast transmission, high infection rates, and infected individuals may be asymptomatic (at initial stage), all of which contribute to the severity of the disease. In parallel with influenza, coronaviruses have the potential of being a seasonal disease.9,10
Vaccine development strategies and platforms
For COVID-19 vaccine development, scientists have done and are doing many efforts. Most of the vaccine developing strategies have used the SARS-CoV-2 S protein as the target region.11 These vaccines are undergoing trials for SARS-CoV-2. Each of these vaccines has their own benefits and drawbacks.12 As of November 8, 2022, 199 vaccine candidates were in preclinical phase, while 172 vaccines were reported to be in clinical development.13
Viral vectored vaccines
Two broad groups of viral vectors are being used for vaccine production: replication-competent and replication-defective viral vectored vaccines. For replication-competent vectors, a lower dose is required to produce a strong immune response, because this vector multiplies resulting in enhanced antigen exposure. Replication-defective vectors are administered in higher dosages as they do not have self-propagation capability. This method is preferred for producing viral vectored vaccines due to its safety. There are various replication-competent and replication-defective COVID-19 candidate vaccines in clinical trials.14 However, only replication-defective counterparts could reach phase III clinical trials. The viral-vector vaccine strategy is detailed in Figure 1a. Viral vector vaccine using non-replicating adenoviral vectors is one of the main vaccine candidates developed. These vectors are engineered with viral genes, especially S protein gene.4 Viral vector vaccines efficiently transfer genes to the target site and induce the immune response of cytotoxic T cells.15 They express a high level of antigenic proteins for longer duration which cause the removal of virus from virus infected cells.16) Though adenoviral vectors have many advantages i.e., scalability, adjuvant qualities and tissue tropism, there are also certain challenges, such as pre-existing immunity in humans that may affect the efficiency of these vectors. Pre-existing immunity against adenovirus type 5 (Ad5) has been reported in humans and, therefore, an alternative adenoviral vector ChAdOx1 has been derived as a vaccine platform with low level of antibodies and T cells in the blood serum.17
Protein subunit vaccines
Protein subunit vaccines that use recombinant antigenic proteins do not induce long-lasting immunity for coronaviruses.18 Vaccines require an adjuvant as an auxiliary support for vaccine-induced immune responses. The function of an adjuvant is to increase the lifespan of the antigenic material or to enhance the cytokine response of the immune system.19 SARS-CoV-2 uses S protein, a transmembrane protein to fuse to the host cell and enter through endocytosis (in certain cells). The S protein fuses with transmembrane protein receptor angiotensin converting enzyme 2, which is present on the surface of human lung tissues.18 This S protein consists of two subunits: S1 and S2. S1 subunit consists of N-terminal domain, receptor binding domain (RBD) and receptor binding motif, while S2 consists of fusion peptide, heptad repeat 1 and heptad repeat 2.20 S glycoproteins possess two conformational states; one is pre-fusion and the other is post-fusion. The antigen used as a vaccine must maintain its pre-fusion state for a good quality of the immune response. The protein subunit strategy is detailed in Figure 1b.21
RNA vaccines
For COVID-19 vaccine, another emerging platform is mRNA, which does not cause any insertional mutagenesis.12 Presently, studies are being conducted on two types of ribosomal nucleic acid (RNA) vaccines: one is replicating and the second is non-replicating RNA vaccines. It is based on chimeric messenger ribosomal nucleic acid (mRNAs) containing the open reading frame sequence of the virus which is not integrated into the chromosome as it is directly translated into the cytoplasm.22 Once mRNA vaccine is injected, the mRNA is processed by the immune cells and, after translation, the production of the target protein activates the immune cells of the body to produce antibodies (neutralizing) to avoid virus entry into cells. The RNA vaccine strategy is detailed in Figure 1c.23
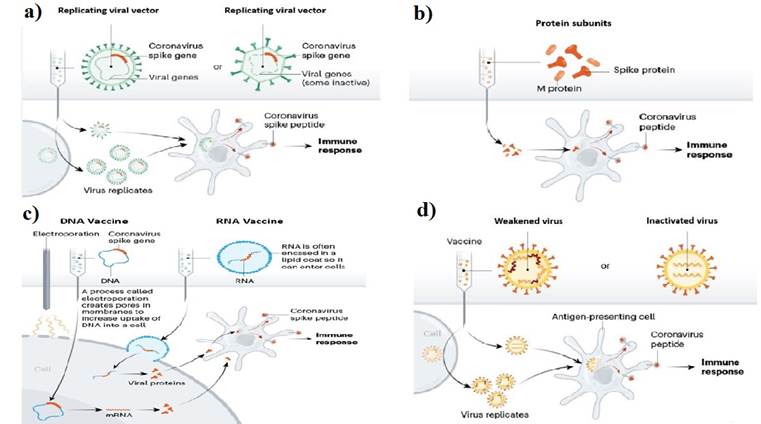
Fig. 1. a) Replicating and non-replicating viral vectore vaccine platform, b) Protein subunit vaccine platform, c) Nucleic acid (DNA/RNA) vaccine platform, d) Live attenuated and inactivated vaccine strategy. Source: (Callaway, 2020)24.
DNA vaccines
To produce enough copies of immunogenic viral proteins, a genetic construct (codes for the specific antigen) is synthetized in deoxyribonucleic acid (DNA) and administered into the host body. DNA vaccines use a plasmid DNA which contains elements such as the DNA sequence of the antigen, the expression elements required for antigen expression in eukaryotes, selectable markers for the selection of the vector containing the gene of interest, followed by a purification process. The eukaryotic expression cassette contains the gene of interest between 5′ promotor and 3′ polyadenine tail that is required for DNA transport into the nucleus, for mRNA stability and its translation inside the infected cell.25 Before entering the nucleus, DNA vaccines must cross two cellular membranes. Different administration methods are used for the introduction of the DNA vector into the body, the most common routes are intramuscular or intradermal injection. There are also other methods of delivery such as gene gun, jet injection and in vivo electroporation, because the previous method of delivery causes low immunogenic response.26
To induce the adaptive immune response, DNA vaccines are administered along with an adjuvant. The antigenic material is first phagocytosed by immature dendritic cells which, through major histocompatibility complex 1 (MHC 1) and MHC 2, present it to CD4+ and CD8+ T cells stimulating humoral and cell-mediated immune responses. The DNA vaccine strategy is detailed in Figure 1c.27
Live attenuated vaccines
In live attenuated vaccines, live pathogens are altered in some way making them less virulent or attenuated before administered into the body. When administered, they provoke the immune response. Its nasal administration is with the help of a nasal spray. An influenza-based vaccine strain with a deletion in the non-structural 1 (NS1) gene, expresses the RBD domain of spike protein, which is present on the surface of SARS-CoV-2. This vaccine is cultivated in chick embryo or Madin Dfarby Canine Kidney (MDCK) cells. Its immunogenic potential is more than that of wild-type influenza virus.12
Inactivated pathogen vaccines
After the production of attenuated cholera vaccine by Pasteur, Salmon and Smith, a new method of vaccine development was introduced by inactivating the pathogen to be used as vaccine to tackle the rare events caused by live attenuated vaccines. The inactivation methods used were heat, chemical treatments (formalin, β-propiolactone), gamma rays.24,28 In inactivated vaccines, a dead form of the pathogen is used, thus confirming the better safety profile compared to live attenuated vaccines. However, sometimes heat, chemicals or radiation cause loss of immunogenicity of the pathogen resulting in less effective form of immunization than attenuated vaccine. Inactivated pathogen vaccines most often require the addition of adjuvants or such compounds that stimulate or amplify immune cells. The inactivated pathogen vaccine strategy is detailed in Figure 1d.14
SARS-CoV-2 vaccine trials
Since the emergence of COVID-19, scientists around the world put their efforts on the development of vaccines. Initial efforts started in China where the outbreak was first reported.12 As of April 6, 2022, more than 196 vaccines were in preclinical and 153 in clinical development, of which 44 vaccines could reach Phase III clinical trials and 10 vaccines, phase IV and are currently being administered to different age groups in different parts of the world. Phase IV clinical trial vaccines are Sinovac, Sinopharm + Beijing institute of Biological Products, AstraZeneca + University of Oxford, CanSino Biologics+ Beijing institute of biological products, Moderna and Pfizer/BioNTech.13 These companies used different vaccine platforms for SARS-CoV-2 vaccines. Sinovac and Sinopharm used inactivated viral vector vaccines in which whole virus is killed; CanSino Biologics, Gamaleya and AstraZeneca used adenovirus vectored platform based on non-replicating or weakened common cold virus; Moderna and Pfizer used the mRNA platform. This mRNA platform is the newest vaccine generation technique in which the synthetic mRNA of antigen is administered into the body of the host to produce the antigen.12,29 Novavax uses protein subunit vaccine in which virus particles are used to stimulate the host immune system.14 Mechanisms of these vaccine platforms are given in Figure 1a, Figure 1b, Figure 1c, and Figure 1d. COVAX is a SARS-CoV-2 vaccines global access facility, together with Gavi, the Vaccine Alliance, Coalition for Epidemic Preparedness Innovations (CEPI) and World Health Organization (WHO). COVAX is a partnership between different world organizations for the development, manufacture and impartial delivery of SARS-CoV-2 vaccine 30. The SARS-CoV-2 vaccine development progress is summarized in Table 2.
Table 2 Vaccine candidates of SARS-CoV-2 and their status.3
Developer | Vaccine | Type | Status |
---|---|---|---|
Sinovac | SARS-CoV-2 vaccine | Inactivated vaccine | Phase IV |
Sinopharm | SARS-CoV-2 vaccine | Inactivated vaccine | Phase IV |
AstraZeneca | ChAdOx1 | Viral vectored vaccine | Phase IV |
CanSino biologics Inc. | Ad5-nCoV | Viral vectored vaccine | Phase IV |
Gamaleya research institute | Sputnik V | Viral vectored vaccine | Phase III |
Janssen (USA) | JNJ-78436725 | Viral vectored vaccine | Phase IV |
Novavax | NVX-CoV2373 | Protein sub-unit vaccine | Phase III |
Moderna | SARS-CoV-2 RNA vaccine | RNA vaccine | Phase IV |
Pfizer/BioNTech | (BNT-162) | RNA vaccine | Phase IV |
Effectiveness of SARS-CoV-2 vaccine
Effectiveness of SARS-CoV-2 vaccine is not simple, but very complex, as its efficacy is checked against multiple parameters like deterrence of symptoms e.g., a person has acquired the virus but will not advance the disease; secondly, preclusion of infection, the efficacy of the vaccine to prevent virus transmission from one person to another and thirdly, protection from severe disease, the efficacy of the vaccine to protect the exposed person from serious disease that requires hospitalization. For each of these parameters, the efficacy is checked after the first and last doses of vaccination against different variants of SARS-CoV-2 (Alpha, Beta, Gamma, Delta and Omicron) (Table 3).31
Vaccine scalability and manufacturing
Vaccine development consists of three stages: antigen discovery which needs series of research, small scale manufacturing of vaccine for clinical trials and after success in full clinical trials, mass production of vaccine in larger scale for worldwide distribution.32 In the current situation, the concerns are not only SARS-CoV-2 vaccine development, but also the supply and manufacturing the vaccine because of considering cost, formulation, and scale-up manufacturing. The demand of the vaccine is high, so in this situation, the developing countries are at a dis-advantageous position. Therefore, platforms and technologies must be considered to minimize the cost of manufacturing vaccine (Table 4).8 Most of the pharmaceutical industries use traditional methods like bioreactors and cell cultures (bacterial, yeast and mammalian) to manufacture vaccine which is highly expensive with high risk of microbial contamination. Therefore, novel methods for rapid vaccine development and manufacturing are now needed. A new approach, plant molecular farming, can be used for the large-scale production of vaccine as it offers scalability: each of the plant acts as a reactor. The greater the number of plants grown, the greater the number of products manufactured. Other characteristics of this approach are low cost and inability of human pathogens to replicate in plant cells.33
Table 4 Production platforms features for vaccine development.
Production platform | Yields | Advantages | Disadvantages |
---|---|---|---|
Mammalian Cells | Moderate to high | High yield Established regulatory approval Correct post-translational modification | High risks of contamination Complex and expensive in development and growth Difficult to scale up |
Bacterial cells | High yields of simple proteins | Well characterized production strains Cheap and simple growth conditions Short production time Established regulatory approval Scalability through fermentation | No human glycosylation Lack of post translational modifications No post-translational modification Large proteins misfolding |
Yeasts | High yields | Short production timescale Cheap and simple growth, conditions Fermentation scalability Proteins correct folding | Bacterial contamination No human glycosylation |
Molecular farming in plants | Moderate to high | Simple scalability with more growth plants Maximum scale up potential Low risks of contamination | Existing regulatory pathway but technology is still very new No human glycosylation |
Insect cells | Moderate to high | Incubation at lower temperature No CO2 required for incubation Higher expression levels when infected with recombinant baculovirus | RNA transcripts inactivation due to cryptic splice sites Expensive on a large scale No human glycosylation |
Vaccine delivery, distribution and administration
Challenges of vaccine administration and delivery depends on the selection of vaccine platform used. The best vaccine platform would have simple integration into devices, designed to be supplied far and wide, manufactured at low cost and administered with feasible supervision. Several vaccine formulations for underdeveloped and developing countries require constant refrigeration; the need for a cold chain hinders the distribution and application of vaccines globally.34 The largest challenge for solution-based vaccines has been the dependence on cold transport. According to WHO, 2.8 million vaccines were lost in five countries due to cold chain failure, and less than 10% countries have effective vaccine management practices. Lyophilized vaccines may be stored at room temperature, but it is difficult to produce such lyophilized solutions.8,35
The most favorable vaccine platform is nanotechnology, derived from bacteriophages and plant viruses, which have evolved as stable nano-containers protecting the genome under various environmental conditions, for example, Cowpea mosaic viruses, that is stable at above 60°C in buffered solutions for about one hour and pH (3.5-9.0) at room temperature.36,37 Furthermore, plant virus nanoparticles are stable under gastrointestinal conditions and orally bioavailable, therefore opening the door for global distribution and oral vaccination. In edible leaf tissue, vaccines could be produced to make possible vaccination of the human population and livestock. Since coronaviruses are zoonotic viruses that can infect humans and animals, this will be good initiative to meet the purpose of health initiative towards the unity of human and veterinary medicines that will be more important to prevent from future outbreaks.38,39
In developing countries, effective vaccination campaigns are required and also access to health care professionals under anomalous circumstance, which is a major challenge during the current global pandemic, where healthcare system does not meet the recommended criteria of WHO.40 Nowadays, suitable alternatives such as film-based vaccines, single dose slow-release implants and micro-needle-based patches are available to address vaccine distribution and their access challenges that could overcome reliance on cold chain and make sure vaccination where health care professionals are rare.40 Microneedles-based patches can be self-administered; such modern vaccine delivery would reduce the burden of healthcare professionals. Melt-processed devices have the advantage of rapid manufacturing at large scale, long term stability and cold chain independence. The potential to ease the burden of medical system and break the cold chain by providing a safe and self-administered prophylactic vaccine has been initialized by different companies such as Zosano, Veleritas Inc., Debiotech and Corium International has led to the filing of more than 10,000 patents globally.41
Conclusion
Since the emergence of COVID-19, scientists worldwide have worked and put their efforts on the development and distribution of vaccines. As of November 8, 2022, 199 vaccines were in pre-clinical phase and 172 in clinical development, of which 49 vaccines could reach the phase III clinical trial and 11 vaccines, the phase IV. Six vaccines are currently being administered worldwide. Most vaccine developing strategies use the SARS-CoV-2 S-protein as the target region.